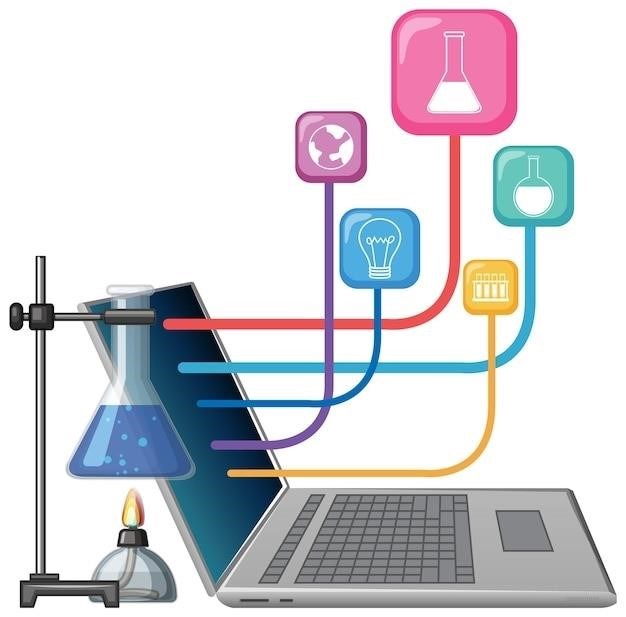
Thermodynamics⁚ An Engineering Approach ― A Comprehensive Overview
Thermodynamics is a fundamental branch of physics that deals with heat, work, temperature, and energy. It is a crucial subject for engineers, as it provides the theoretical foundation for understanding and designing a wide range of engineering systems. This comprehensive overview will delve into the core concepts of thermodynamics, explore its significance in various engineering disciplines, and illuminate its enduring impact on technological advancements.
Introduction to Thermodynamics
Thermodynamics is the study of how energy is transferred and transformed in physical systems. It is a fundamental science that underpins many engineering disciplines, including mechanical, chemical, and aerospace engineering. The core principles of thermodynamics govern the behavior of energy in various forms, such as heat, work, and internal energy. These principles are applied to analyze and design engines, power plants, refrigeration systems, and many other engineering systems.
Thermodynamics is based on a set of fundamental laws that describe the behavior of energy in physical systems. These laws are universal and apply to all systems, regardless of their size or complexity. The first law of thermodynamics states that energy cannot be created or destroyed, only transferred or transformed. The second law of thermodynamics states that the entropy of a closed system always increases over time. The third law of thermodynamics states that the entropy of a system approaches a constant value as the temperature approaches absolute zero.
Thermodynamics is a powerful tool for understanding and predicting the behavior of engineering systems. It is used to design efficient engines, develop new materials, and optimize processes in a wide range of industries.
The Importance of Thermodynamics in Engineering
Thermodynamics plays a pivotal role in engineering, providing the theoretical framework for understanding and optimizing energy conversion processes. Its principles are essential for designing and analyzing systems that involve heat transfer, work, and energy transformations. Engineers rely on thermodynamics to optimize the efficiency of power plants, design efficient engines, and develop innovative refrigeration and air conditioning systems.
Thermodynamics is crucial in various engineering fields. In mechanical engineering, it guides the design of engines, turbines, and other machinery that convert heat into mechanical work. In chemical engineering, it is used to analyze and optimize chemical reactions, design reactors, and develop new materials. In aerospace engineering, thermodynamics is essential for understanding the performance of aircraft engines, rockets, and spacecraft.
The principles of thermodynamics are also applied in other areas, such as environmental engineering, where they are used to assess the environmental impact of energy production and consumption. Understanding thermodynamics is essential for developing sustainable technologies and mitigating the effects of climate change.
Key Concepts in Thermodynamics
Thermodynamics is built upon a set of fundamental concepts that define its scope and provide the basis for its applications. These concepts are essential for understanding the behavior of systems involving heat, work, and energy. Some of the key concepts in thermodynamics include⁚
- System⁚ A system is a defined region of space or a collection of matter that is being studied. It can be open, closed, or isolated, depending on its interaction with the surroundings.
- Surroundings⁚ The surroundings are everything outside the system that can interact with it.
- Boundary⁚ The boundary separates the system from the surroundings and defines the limits of the system.
- Properties⁚ Properties are characteristics of a system that can be measured or calculated, such as pressure, temperature, volume, and mass.
- State⁚ The state of a system is defined by the values of its properties. A change in state occurs when one or more properties change.
- Process⁚ A process is a series of changes in the state of a system. A process can be reversible or irreversible, depending on whether it can be reversed without leaving any trace on the surroundings.
- Equilibrium⁚ A system is in equilibrium when its properties are not changing with time. There are different types of equilibrium, such as thermal equilibrium, mechanical equilibrium, and chemical equilibrium.
These key concepts provide the foundation for understanding and applying thermodynamics in various engineering applications.
Thermodynamic Systems and Their Properties
Thermodynamic systems are the fundamental entities studied in thermodynamics. They are defined regions of space or collections of matter that are isolated for analysis. The behavior of these systems is governed by their properties, which are measurable characteristics that describe the system’s state. Understanding the different types of systems and their properties is crucial for applying thermodynamic principles to engineering problems.
- Open System⁚ An open system can exchange both energy and mass with its surroundings. Examples include a boiling pot of water or a combustion engine.
- Closed System⁚ A closed system can exchange energy with its surroundings but not mass. A sealed container of gas is an example.
- Isolated System⁚ An isolated system cannot exchange either energy or mass with its surroundings. A perfectly insulated container is an idealized example.
Each system type has unique properties. These properties can be extensive, meaning they depend on the system’s size (e.g., volume, mass), or intensive, meaning they are independent of size (e.g., pressure, temperature). The relationships between these properties are fundamental to thermodynamic analysis.
The First Law of Thermodynamics
The First Law of Thermodynamics, often referred to as the law of conservation of energy, is a fundamental principle that governs energy transformations within a system. It states that energy cannot be created or destroyed, only transformed from one form to another. This law has far-reaching implications in engineering, particularly in the analysis of energy conversion systems, such as power plants and engines.
The First Law can be expressed mathematically as⁚
ΔU = Q ― W
Where⁚
- ΔU is the change in internal energy of the system.
- Q is the heat added to the system.
- W is the work done by the system.
This equation highlights the key aspects of the First Law. The internal energy of a system can be increased by adding heat or decreased by the system doing work. The First Law underscores the importance of energy balance in engineering applications.
The Second Law of Thermodynamics
The Second Law of Thermodynamics introduces the concept of entropy, a measure of disorder or randomness within a system. It states that the entropy of an isolated system always increases over time. In simpler terms, this law implies that processes naturally tend to move towards a state of greater disorder, and it is impossible to create a process that is 100% efficient in converting heat to work.
The Second Law has profound implications for engineering, particularly in the design and analysis of thermodynamic cycles, such as those found in power plants and refrigeration systems. It sets limits on the efficiency of energy conversion processes, reminding engineers that there will always be some energy lost as heat during any energy transformation. This law also helps explain why certain processes are irreversible and why perpetual motion machines are impossible to create.
The Second Law can be expressed in various ways, including the Kelvin-Planck statement, which states that it is impossible to create a device that continuously converts heat into work without any other effect, or the Clausius statement, which states that it is impossible to transfer heat from a colder body to a hotter body without external work.
The Third Law of Thermodynamics
The Third Law of Thermodynamics establishes the absolute zero of temperature as an unattainable limit. It states that the entropy of a perfect crystal at absolute zero is zero. This means that at absolute zero, a system reaches its most ordered state with no thermal energy and no randomness. However, absolute zero is a theoretical concept, and it is impossible to achieve in practice.
The Third Law has significant implications for understanding the behavior of matter at extremely low temperatures; It provides a theoretical framework for studying phenomena like superconductivity and superfluidity, where materials exhibit unusual properties at very low temperatures. It also serves as a foundation for developing advanced technologies, such as cryogenic engineering, which involves the study and application of extremely low temperatures.
The Third Law is often used in conjunction with the First and Second Laws to calculate the entropy of a system at a particular temperature and pressure. It also highlights the importance of entropy as a fundamental property of matter, impacting the behavior of systems and influencing the design of various engineering processes.
Thermodynamic Processes and Cycles
Thermodynamic processes refer to changes in the state of a system, characterized by variations in properties like pressure, temperature, and volume. These processes can be classified as isothermal, adiabatic, isobaric, or isochoric, based on the specific conditions maintained during the change. For example, an isothermal process occurs at constant temperature, while an adiabatic process involves no heat transfer.
Thermodynamic cycles represent a series of interconnected processes that ultimately return the system to its initial state. These cycles are crucial in engineering applications, as they form the basis for various devices like heat engines, refrigerators, and power plants. For instance, the Carnot cycle, a theoretical cycle with maximum efficiency, provides a benchmark for analyzing the performance of real-world engines.
Understanding thermodynamic processes and cycles is essential for engineers to analyze the efficiency of energy conversion systems, optimize the performance of engines and power plants, and develop innovative technologies for energy production and utilization. The study of these processes and cycles provides a framework for designing and improving various engineering systems, contributing to advancements in energy efficiency and sustainability.
Applications of Thermodynamics in Engineering
Thermodynamics finds extensive applications across various engineering disciplines, shaping the design and operation of countless technologies. In mechanical engineering, thermodynamics is fundamental to understanding and optimizing engines, power plants, and refrigeration systems. The principles of heat transfer, work, and energy conversion are crucial for designing efficient and sustainable energy systems.
Chemical engineers rely on thermodynamics to analyze chemical reactions, optimize process efficiency, and design reactors. The study of chemical equilibrium, reaction kinetics, and phase changes enables the development of efficient chemical processes for manufacturing, energy production, and environmental remediation.
Thermodynamics also plays a vital role in aerospace engineering, where it is applied to optimize rocket propulsion systems, design aircraft engines, and analyze the thermal behavior of spacecraft. Understanding heat transfer, fluid mechanics, and combustion processes is critical for ensuring the safety and efficiency of aerospace vehicles.
Thermodynamics in Power Generation
Thermodynamics forms the bedrock of power generation, underpinning the conversion of energy from various sources into electricity. Power plants, whether fueled by fossil fuels, nuclear fission, or renewable sources like solar and wind, rely on thermodynamic principles to achieve efficient energy conversion. The core concept of the Carnot cycle, a theoretical thermodynamic cycle, provides a benchmark for efficiency in power generation.
In thermal power plants, the combustion of fuels generates heat, which is then used to produce steam. This steam drives turbines, converting thermal energy into mechanical energy. The turbines are coupled to generators, transforming mechanical energy into electrical energy. The efficiency of this process is governed by thermodynamic principles, including the first and second laws of thermodynamics, which dictate the limits of energy conversion and the inevitable loss of energy as heat.
Furthermore, thermodynamics plays a crucial role in the design and optimization of power plant components, such as boilers, turbines, and condensers. Understanding heat transfer, fluid dynamics, and combustion processes is essential for maximizing efficiency and minimizing environmental impact.
Thermodynamics in Refrigeration and Air Conditioning
Refrigeration and air conditioning systems rely heavily on thermodynamic principles to achieve their primary functions⁚ cooling and temperature control. These systems operate based on the concept of heat transfer and the movement of heat from a colder region to a warmer region, a process that requires energy input. The core thermodynamic cycle employed in refrigeration and air conditioning is the vapor-compression refrigeration cycle, which involves four key stages⁚ evaporation, compression, condensation, and expansion.
In the evaporation stage, a refrigerant absorbs heat from the cold space, causing it to vaporize. The refrigerant vapor is then compressed, increasing its temperature and pressure. In the condensation stage, the hot refrigerant vapor releases heat to the surroundings, condensing back into a liquid. Finally, the refrigerant expands, decreasing its pressure and temperature, preparing it for the next cycle of heat absorption. The efficiency of this cycle is determined by the refrigerant used, the design of the components, and the operating conditions.
Thermodynamics is essential for understanding the energy requirements, performance, and optimization of refrigeration and air conditioning systems. It helps engineers design systems that are efficient, environmentally friendly, and meet specific temperature control needs.
Thermodynamics in Chemical Engineering
Chemical engineering is deeply intertwined with thermodynamics, as it governs the energy balance and feasibility of chemical reactions, separation processes, and material transformations. Thermodynamics provides a framework for understanding the energy changes that occur during chemical reactions, allowing engineers to predict the equilibrium conditions and optimize reaction yields. It also enables the analysis of heat transfer and mass transfer processes, which are crucial in chemical processing.
Key applications of thermodynamics in chemical engineering include⁚
- Reaction Equilibrium and Kinetics⁚ Thermodynamics helps determine the equilibrium constant of a reaction, predicting the extent to which a reaction will proceed under given conditions.
- Process Design and Optimization⁚ Thermodynamics is used to design and optimize chemical processes, such as distillation, extraction, and crystallization, ensuring efficient energy utilization and maximizing product yields.
- Thermochemical Analysis⁚ Thermodynamic principles are applied to analyze the energy changes associated with various chemical reactions and processes, enabling the prediction of heat release or absorption and the selection of suitable materials for process equipment.
Thermodynamics plays a vital role in ensuring safe, efficient, and sustainable chemical engineering processes, contributing to the development of new technologies and materials.
The Enduring Significance of Thermodynamics
Thermodynamics stands as a cornerstone of engineering, providing a robust framework for understanding and manipulating energy transformations. Its principles are woven into the fabric of countless technologies, from power generation and refrigeration to chemical synthesis and material science. By elucidating the fundamental relationships between heat, work, and energy, thermodynamics empowers engineers to design efficient, sustainable, and innovative systems.
The enduring significance of thermodynamics lies in its ability to predict and control energy flows, ensuring that engineering solutions are both effective and environmentally responsible. As technological advancements continue to shape our world, the principles of thermodynamics will remain indispensable in driving innovation and addressing the challenges of a rapidly evolving global landscape.
From the design of efficient power plants to the development of novel energy storage solutions, thermodynamics remains a fundamental pillar of engineering, guiding the creation of a more sustainable and energy-conscious future.